Author: Dr. Suresh Kaushik
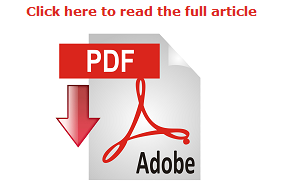
Size: 360 KB
INTRODUCTION
Biodiversity is generally defined as the variety and variability of living organisms and the ecosystems in which this occurs. The variability of life in the soil encompasses not only plants and animals but also the invertebrates and microorganisms that are interdependent on one another and the higher plants they support. Biodiversity is composed of three interrelated elements: genetic, functional and taxonomic diversity as shown in Figure 1. Taxonomic diversity i.e. the number of species forms an important part of an ecosystem’s diversity and is controlled by the genetic diversity. Genetic diversity can be much more than the number of recognized species. Hence, several species may have the same functions, resulting in functional redundancy. Some species may also interact to perform functions not possible by any single species. Therefore, biodiversity is the interaction of all these elements. Soil biodiversity is more extensive than any other environment on the globe when all living forms are considered. The soil biota contains representations of all groups of microorganisms, fungi, bacteria, algae and viruses, as well as the microfauna such as protozoa and nematodes. The total diversity is equal to greater than any coral reef or rain forest. Soil algae and protozoa, like higher plants and animals, can be identified by their morphology. Fungi and bacteria, however, require more extensive biochemical and genetic analysis to enable identification. It has been estimated that only between 1 and 5% of all microorganisms on the earth have been named and classified. A large proportion of these unknown species is thought to reside in the soil. The possible numbers of existing species of different groups are 1.5 million species of fungi, 300,000 species of bacteria, 400,000 species of nematodes and 40,000 species of protozoa. New molecular techniques have been used to estimate that single gram of soil probably contains several thousand bacterial species.
SOIL FUNCTIONS
Soil functions are essential for the biosphere but the soil is perhaps the most difficult and poorly understood environment of all. The main ecological functional of soil include supply of nutrients to support plant growth; buffering and transformation of potentially harmful compounds and elements i.e. pesticides and heavy metals; exchange of gases and waters between atmosphere and hydrosphere, and biological habitat of species and genes. All these functions are to a lesser or greater extent dependent on the activity of microorganism. Soil microorganisms provide these functions by a combination of their functional attributes and the manner in which they form an interactive food web. The activity of soil microorganism is responsible for the colour, structure and even the smell of soil. A volatile chemical â€" geosmin â€" is produced by a group of filamentous bacterial- the actinomycetes after soil disturbance and is responsible for the earth odour. Bacterial have diverse metabolic capacities that allow them to exhibit the wide range of energy sources in soil. Along with fungi, they are the primary agents of biogeochemical transformations and the decomposition of dead plant and animal remains which recycle nutrients for plant growth (Fig.2). Practically every chemical compounds, both natural and manufactured, ends up in the soil and we rely on the soil to retain and transform these compounds, thereby protecting surface and ground waters. Soil contributes to the regulation of the global atmosphere. The gaseous end products of microbial activity include carbon dioxide, methane, nitrous oxide, some of which contribute to the greenhouse effect responsible for global warming. Some bacteria and fungi form important symbiotic associations with plants, for instance, Rhizobium bacteria form nitrogen-fixing nodules in legumes in mycorrhiza (root dwelling fungi) live in symbiosis with nearly all plants in the natural environment. Mycorrhiza helps plants in the uptake of essential nutrients. They also protect the plant from pollutants and plant pathogens. Nematodes and microfauna, especially protozoa, play important role in nutrient cycling. They act as predators of the microflora and organic detritus and releasing nutrients back to plants. Mesofauna and macrofauna, in turn act as predators of microfauna, creating an interdependent food web responsible for recycling of essential elements for dead organic matter living organisms.
CAUSES FOR SOIL BIODIVERSITY
Soil microbial diversity and functioning is a product of soil, climate and plant factors. Soils exhibit a high degree of spatial heterogeneity both vertically and horizontally. Soil properties can vary within fields and is a complex network of pores ranging in size from 0.2 micron to 2 millimeter. Thus, many of the smallest organisms are protected from predation in those pores. Another reason for biodiversity can be variation in many of the important chemical and physical properties of soil such as pH and oxygen status over a short distance e.g. from rhizosphere to bulk soil and from inner to outer surfaces of a soil crumb. The spatial heterogeneity of potential living spaces, the diversity of food and energy sources available and temporal variation in moisture, all lead to cause the biodiversity.
QUANTIFICATION OF SOIL BIODIVERSITY
The measuring of soil microbial diversity has traditionally been problematic due to the vast numbers of potential species and the difficulties of culturing organisms in the lab using traditional techniques. In microorganisms, especially bacteria, there is an additional problem with taxonomy because of continuous exchange of DNA from one species to another, so that the species concept may be invalid. Biodiversity in soil is not simply about evaluating species numbers but includes evaluating the way species interact with one another and their environment. In order to measure the impact of changing community structure, biodiversity must be linked with measures of ecosystem function. Chemical compounds found in microbial cell walls, such as phospholipids fatty acids, can be extracted. These compounds are specific to certain types of microbes and can provide information regarding broad changes in community structure. There is an enormous reservoir of uncultured bacteria, many of which are only discovered and described by their DNA sequences. The bacteria that can be cultured are only a small fraction (1-10%) of the numbers actually contained in soil. Many cells in soil are in a viable but non-culturable state. Molecular techniques have shown that they are metabolically active but do not divide and grow. This represents one of the greatest challenges to quantify the diversity of soil bacteria. New molecular techniques (Figure 3) can be used to measure genetic diversity of soil organism without the need to isolate and culture them in the lab. DNA directly from soil and determining DNA sequence will eventually lead to test kits that can identify and quantify such organisms in situ. The challenge at present is to disentangle the vast amount of genetic material extracted from soils.
MOLECULAR TECHNIQUES TO MEASURE SOIL MICROBIAL DIVERSITY
Culture based studies, which require the growth of microorganism in the laboratory, are severely limited by the fact that less than 1% of soil microorganisms can be cultured [1]. Culture-based studies thus provide an extremely restricted view of the diversity of the natural microflora. Advances in molecular techniques have led to significant advances in the study of soil microbial communities (Tab.1). The molecular approaches are mainly based on the RNA of the small ribosomal subunit (16S rRNA for prokaryotices) or their corresponding genes, considering it as a “molecular clock”. This molecule was chosen because it presents some specific features, such as its universal distribution among all organisms, and some highly conserved and other highly variable regions. This allows comparison of organism within the same domain, as well as differentiation of strain of same species. Profiles for microbial communities based on differences in the 16S rRNA gene sequences of their constituents provide insight into differences in microbial population structure over time or with different experimental treatments.
Therefore, it is possible to make a comprehensive survey of the microbial diversity of a natural habitat in a relatively simple and more extensive way than provided by cultivation techniques. The molecular techniques that are commonly used to assess soil microbial communities are described here.
APPROACH BASED ON 16S RIBOSOMAL RNA
This approach based on small subunit ribosomal RNA (SSU rRNA) has provided a comprehensive phylogenetic framework for the analysis of microbial communities [2]. The 16S rRNA gene (Fig.4) is a useful phylogenetic marker because
• It is present in all organisms from bacteria to higher organism, and its sequence is fairly well conserved across phylogenetic groups.
• Its sequences differ between species.
• Some sections of the sequence are highly conserved , whereas others differ greatly among organisms, showing evolutionary relationship between organism.
• Its sequences are available on databases to use for identification purposes.
The 16S Ribosomal RNA Molecule: The 16S rRNA molecule is a major component of the small ribosomal subunit (Fig.4). It has approximately 1500 ribonucleotides (Fig.4). This single-stranded rRNA molecule has an intricate secondary structure with extensive intra-chain base pairing. The 16S rRNA forms a part of the ribosomal structure that is the site of protein biosynthesis resulting in the translation of messenger RNA. The 3' end of the bacterial 16S rRNA base-pairs with the Shine-Dalgarno sequence located upstream of the AUG initiation codon in mRNA during the initiation step of the translation process. This allows the mRNA to position itself on the ribosome. There is also evidence that 16S rRNA is directly involved in the interactions between the large and small ribosomal subunits. The sequence of 16S rRNA is highly conserved among all organisms due to the antiquity of the protein-synthesizing process. Thus ribosomal RNA is an excellent molecule for discerning evolutionary relationships among prokaryotic organisms. Ribosomal RNAs are ancient molecules, functionally constant, universally distributed, and moderately well conserved across broad phylogenetic distances. Various regions within the rRNA genes evolve at slightly different rates due to the fact that 16S rRNA is functionally involved in the protein biosynthesis process and involved in different interactions in the ribosome. This results in alternating regions in the rRNA sequences of nucleotide conservation and variability. The 16S rRNA of most major phylogenetic groups has one or more characteristic nucleotide sequences called oligonucleotide signatures. Oligonucleotide signature sequences are specific sequences that occur in most or all members of a particular phylogenetic group. Because the number of different possible sequences is so large, similarity in two sequences always indicate some phylogenetic relationship. However, it is the degree of similarity in the sequences between two organisms that indicates their relative evolutionary relatedness. Thus signature sequences can be used to place microorganisms in the proper group. Molecular techniques that analyse prokaryotic SSU rRNA (16S rRNA) have been applied widely to the study of microbial community structure in soils. A variety of methods can be used for the analysis of soil microbial communities via 16S rRNA.
EXPLORING THE UNCULTIVABLE BACTERIAL WORLD
The first and fundamental step to explore molecular microbial ecology is to obtain the nucleic acids (DNA or RNA). There are different protocols available in literature. The choice of which protocol to use for each approach will depend on many variable such as type of sample, type of subsequent analysis, and the quality and quality of nucleic acids recovered from environmental samples will be critical for the success of any molecular study. Subsequent molecular biology techniques (Figure 5)most often applied to study soil microbial biodiversity and ecology are given below:
POLYMERASE CHAIN REACTION -BASED TECHNIQUES
PCR is used to amplify a sequence of DNA using a pair of oligonucleotide primers each complementary to one end of the DNA target sequence. These are extended towards each other by a thermostable DNA polymerase in a reaction cycle of three steps: denaturation (95°C), primer annealing (55°C) and polymerization (72°C) as shown in figure 6.
Sequencing of amplified 16S rRNA gene: The Polymerase Chain Reaction (PCR) is used to amplify all of the 16S rRNA genes within a sample and a phylogenetic inventory of the prokaryotic component of a soil microbial community can be assembled. This collection of amplified genes subsequently cloned and sequenced. Universal primers designed to amplify all of the 16S rRNA genes within a sample [3]or primers designed to amplify the 16S rRNA genes of a specific group e.g. ammonia oxidizing bacteria [4]. Phylogenetic inventories produced by such method have revealed much greater diversity in soil microbial communities than was detected by classical culture-based studies and thus significantly increased our understanding of soil biological diversity. However, these inventories are extremely laborious to produce and are therefore always incomplete as it is not practical at this stage to sequence every amplicon produced for PCR [5].
Denaturing Gradient Gel Electrophoresis (DGGE)
Denaturing Gradient Gel Electrophoresis (DGGE) is a method only recently introduced into molecular biology [6]. This profiling technique is based on the melting behaviour of the 16S rRNA gene on a polyacrylamide gel containing a urea gradient, which is dependent on the G+C content and the nucleotide sequence. The primers used to amplify the 16S rRNA genes in the community contain a GC clamp; as the DNA fragment moves down the gradient it will denature except for the terminal GC clamp (Fig. 4). The denaturation or melting dramatically reduces the mobility of the DNA fragment in the gradient gel. Amplification of the 16S rRNA genes within a community and subsequent analysis by DGGE gives rise to a banding pattern in which each band corresponds to a single species. The advantage of this technique is that the band may be recovered from the gel for further analysis such as sequencing (Fig. 7). It has been shown to detect differences in the denaturing behaviour of small DNA fragments (200-700bp) that differ by as little as one base pair. In normal gel (agarose or acylamide) electrophoresis, DNA fragments are separated by size. As the size of the DNA fragment increases, it velocity through the gel decreases. In DGGE, DNA fragments of the same size are separated by their denaturing profile i.e. how the dsDNA becomes ssDNA. When dsDNA is subjected to an increasingly denaturing physical environment, it partially melts. As the denaturing environment increases, the DNA fragment will dissociate. This physical denaturing of the DNA fragment does not proceed in a zipperlike manner. As the DNA fragment enters the denaturing conditions, discrete portions of the fragment will denature. As a result of this ds to ss conformational change, the DNA fragment's passage through the gel is drastically slowed. The dsDNA fragment will travel faster than a denatured (ss) DNA fragment. As the ds DNA fragment is electrophoresed through a gradient of increasing deanturant, it partially melts and undergoes both a conformational and mobility change. A constant temperature (of around 60°C) and chemical denaturants (100% Denaturant is 40% formamide and 7M Urea) are used in practice.
Temperature Gradient Gel Electrophoresis (TGGE)
Temperature Gradient Gel Electrophoresis (TGGE) provides a temperature gradient instead of a chemical gradient. This is another method that relies upon the conformational change of dsDNA to ssDNA to separate equal size fragments. The position in a gel where the dsDNA fragment melts and becomes ssDNA (and almost stops) is dependent on the nucleotide sequence in the melted region. Different sequences will result in different positions in the gel where the DNA fragment halts. This method is powerful in separating same size DNA fragments or PCR products based on sequence. In studying environmental microbial communities, one gene, the 16S rRNA gene, is amplified from the extracted DNA from that whole community. This gene is ideal because there is a large database (the Ribosomal Database Project), it is ubiquitously present throughout life, it is rarely laterally transferred (it is species specific) and its function is conserved. It is used as a phylogenetic aid in identifying and relating different sequences.
Terminal Restriction Fragment Length Polymorphism (T-RFLP)
Terminal restriction fragment length polymorphism (T-RFLP) analysis is a method for rapid profiling of mixed populations of an homologous amplicon (i.e., diverse sequences of a single gene). It involves tagging one end of PCR amplicons through the use of a fluorescent molecule attached to a primer. The amplified product is then cut with a restriction enzyme. Terminal restriction fragments (T-RFs) are separated by electrophoresis and visualized by excitation of the fluor [7]. T-RFLP analysis provides quantitative data about each T-RF detected, including size in base pairs and intensity of fluorescence (peak height). T-RF sizes can be compared to a database of theoretical T-RFs derived from sequence information. T-RFLP profiles have been shown to be relatively stable to variability in PCR conditions. In most cases, both the sizes and relative signal intensities of the individual T-RFs in a sample are highly reproducible. Consequently, TRFLP analysis is an excellent tool for rapidly comparing microbial communities. Assessment of the diversity, structure, and dynamics of complex microbial communities with T-RFLP has mainly been based on PCR-amplified 16S rRNA genes (16S rDNA). Being a community profiling method based on the 16s rRNA gene, can be used with universal down to species level primers depending on the resolution required. This method however does require amplification of the 16s gene with specific primers and is thus more susceptible to biases and skewing of the native community. The technique itself depends on the amplification of the DNA with a primer set, one with a fluorescently end labeled primer and restriction of the resulting product with frequently cutting enzymes. Due to sequence variations the terminal restriction site for each species in the community should be different. The output is digital and provides information on the size of the product in base pairs (i.e. species) and the intensity of fluorescence or relative abundance of the various community members.
PROBE-BASED TECHNIQUES:
In additions to PCR-based techniques, probe based methods can be used to detect specific phylogenetic groups of bacteria in soil. DNA probes are composed of short segments of DNA which can be designed to target regions of the 16S rRNA gene that are unique to a particular phylogenetic group and can be synthesized in the laboratory [8]. These probes then can be hybridized to RNA extracted from a soil sample and provide information on the presence or absence, as well as relative abundance of that group targeted by that probe. Since active cells contain thousands to tens of thousands of copies of ribosomal RNA per cell. Therefore, ribosomal RNA is a good target for probing technology, thus making it a naturally amplified target [9,10]. The application of DNA probes is generally carried out using membrane-based [11, 12] or in situ hybridization techniques. The FISH uses in situ hybridization of probes. Fluorescent In Situ Hybridization (FISH) The 16s rRNA is targeted in situ by a probe that has been labeled with a dye that fluoresces under a particular wavelength of light e.g. TAMRA which fluoresces red at 575nm. The probe may be general such as the universal bacterial probe EUB 338 or may be specific to family, genus or even species level depending on the questions being asked. The bacteria are then visualised under a fluorescent microscope or if more information is required, for example the construction of a 3D image, confocal scanning electron microscopy may be used.16S rRNA-based approaches have provided a wealth of information on soil microbial composition, but these approaches have some limitations. Although these approaches can indicate which phylogenetic groups of microbes are present in a soil sample, but they provide no information on the metabolic processes being carried out by those groups. In addition, group-specific 16S rRNA approaches may not be suitable for analysis of specific functional guilds if the function is distributed widely over the phylogenetic tree.
APPROACH BASED ON FUNCTIONAL GENES
The analysis of genes encoding enzymes involved in specific metabolic functions (functional genes) can overcome some of the limitations of 16S rRNA-based techniques. Several functional genes have been discovered that are well conserved across phylogenetic groups e.g. the genes coding for nitrogenase (the enzyme that catalyses biological nitrogen fixation) [13], ammonia monoxygenase (the enzyme that involves in nitrification) [14] and nitrite reductase (the enzyme that involves in denitrification) [15]. Because these genes are conserved across phylogenetic groups, so it more practical choice for the study of these groups than 16S rRNA. In addition, functional genes often provide a level of resolution below specifies because functional molecules may experience higher rates of evolutionary changes than the 16S rRNA molecule. Functional genes can be used to assess the diversity of functional guilds in several ways. Once a functional gene has been identified, the diversity of this gene in different microbial species can be analysed by PCR-based approaches. The diversity of functional genes can be assessed directly from the environment. Specific PCR primers can be used to amplify the target gene from DNA extracted from environmental samples. This mixture of functional gene amplicons can be used to create a clone library, and individual clones can be sequences and compared. Similarly, the diversity of environmentally derived functional gene amplicons can also be assessed by profiling techniques such as T-RFLP [16] and DGGE [17]. The T-RFLP profiles obtained from the environmental samples enabled the researchers to rapidly assess the complexities of ammonia oxidizing community in the environmental samples. There has also been development of DNA probes targeting functional genes e.g. nir gene (nitrite reductase- the enzyme which involves in denitrification) [18]. The application of DNA probes is generally carried out using membrane-based or in situ hybridization techniques. These techniques can be used for probes targeted to various functional genes. However, because these hybridization techniques severely limit the number of probes that can be applied simultaneously. So the amount of acquired information is limited.
DNA Microarrays
DNA microarrays offer a much higher probe capacity. DNA microarrays generally consist of a set of nucleic acids that are spotted (Figure 8) and covalently bound to some solid support, such as a glass slide [16]. On a typical DNA microarrays, hundreds to tens of thousands of nucleic acids can be spotted within a very small surface area, can be hybridized simultaneously [17]. In addition to higher probe capacity, microarrays also offer the advantages of increased speed of detection, low cost and the potential for automation [18]. This technology has been used for characterizing entire genomes as well as for measuring gene expression [19]. This technology has great potential for characterizing microbial communities and their function in the environment. However, the application of DNA microarrays to the assessment of microbes in the environment poses a number of technical challenges, including optimization of probe-target specificity and quantification of target genes. Recent work has demonstrated that these technical challenges can be overcome. Based on the potential advantages of DNA microarrays and recent technical advances, it is likely that the application of 16S rRNA targeted DNA microarrays environmental systems will increase dramatically in the near future.
APPROACH BASED ON FUNCTIONAL GENE EXPRESSION
The presence of functional genes by PCR-based techniques can be assessed as described above, but it is possible now to assess the expression of functional genes in the environmental samples by the detection of mRNA. Since mRNA is critical intermediate in the gene expression and has a short half-life. Detection of mRNA specific for a gene is a strong indicator that the gene is being actively converted to protein [20]. In addition, the number of mRNA transcripts is correlated with level of activity [21]. Several different techniques have been employed to assess functional gene expression by detecting mRNA.
Reverse Transcriptase-PCR (RT-PCR)
RT-PCR is an effective tool for mRNA detection. In this, mRNA is used as a template to synthesize a complementary strand of DNA (cDNA). This cDNA is then amplified by PCR. The amplicons can be cloned and sequenced. This powerful analysis method could be an excellent technique for the analysis of functional guilds in soils. Detection of mRNA can also be done via DNA probes and conventional hybridization techniques. Probes targeted to mRNA can also be applied in a DNA microarrays format. So far the use of microarrays to monitor gene expression has been confined largely to analysis of expression patterns in a single organism (usually E. coli). But recently, DNA microarrays for the detection of expression in complex microbial communities has been employed. A DNA microarrays that included 64 functional genes from a variety of organism was built [22]. This technique shows great promise for the monitoring of gene expression in soils.
SIGNIFICANCE OF SOIL BIODIVERSITY
Soil biodiversity is probably the largest component of total biodiversity. It is highly probable that many habitats of nature conservation value are sustained and maintained by soil biodiversity. Although we are still unsure of the links to ecosystem functioning and above ground biodiversity. Soil microorganisms are vital to sustaining the biosphere and functioning of ecosystems and consequently, can be used for maintaining and predicting environmental change and pollutant impacts. Since soil functioning depends on the activity of microorganisms, measures of the activity, biomass and diversity of soil microorganisms have been proposed as indicators of soil quality. Before doing this, we should be able to link biological and chemical parameters to predict changes in soil functioning as microbial biomass is usually proportional to levels of soil organic matter. Therefore, using the ratio of biomass to soil organic matter or to the rate of decomposition may provide more sensitive and meaningful ways to assess change. The untapped diversity of microorganisms is a resource for new genes and species that may have value to biotechnology and medicines. Microorganisms have been the main source of medicinal compounds such as antibiotics, immunosuppressants, anti-tumor agents and pesticides, vitamins, enzymes etc. Hence, the vast unexplored biodiversity of soil has potential for commercial exploitations in biotechnology especially in areas of medicines, agriculture, industrial processes and bioremediation of polluted wastes, waters and land. Thus, by understanding the complexity and limits of life, especially in extreme environment, we can expand our knowledge regarding the diversity and extent of life in general and the possibilities of life on other planets such as Mars.
CONCLUSIONS:
Little is known about soil biodiversity as compared to other environments, even though terrestrial ecosystem cannot function without it. Now it is possible to unlock some of the secrets of the soil which is not possible earlier. Molecular techniques have revolutionized the field of soil microbiology over the last 25 years. Application of these techniques to study of 16S rRNA genes has created a comprehensive framework for microbial phylogeny and has dramatically expanded our understanding of soil microbial diversity. Recent functional gene studies have already contributed to our understanding of functional guild diversity. Functional gene analysis techniques will ultimately allow for effective monitoring of the expression of these genes in soil. An exciting new technology, DNA microarrays, has already demonstrated its utility as format for the application of both 16S rRNA and functional gene targeted DNA probes. Because of these high probe capacity, DNA microarrays offers the potential to build complex array that integrate monitoring of both 16S rRNA and functional genes, which will enables us to examine both phylogenetic and functional components of soil microbial communities. Therefore, molecular techniques such as DGGE, T-RFLP, and DNA microarrays in particular, should help to revolutionize our understanding of the complex role that microorganisms play in soil processes.
REFERENCES
1. Amann, R.I., W. Ludwig, and K.H. Schleifer. Microbiol. Rev., 1995, 59:143-169.
2. Macrae, A., Braz. J. Microbiol., 2000, 31:77-82
3. Borneman, J., P.W. Skroch, K.M. O’Sullivan, J.A. Palus, N.G. Rumjanek, J.L. Jansen, J. Nienhuis, and E.W. Triplett, Appl. Environ. Microbiol., 1996, 62:19321943.
4. Kuske, C.R., S.M. Barns, and J.D. Busch, Appl. Environ. Microbiol., 1997, 63:3614-3621
5. Bruns, M. A., J.R. Stephen, G.A. Kowalchuk, J.I. Prosser, and E.A. Paul, Appl. Environ. Microbiol. 1999, 65:2994-3000.
6. Tiedje, J.M., S. Asuming-Brempong, K. Nusslein, T.L. Marsh, and S.S. Flynn. Appl. Soil Ecol., 1999, 13:109-122.
7. Kowalchuk, G.A., P.L.E. Bodelier, G.H.J. Heilig, J.R. Stephen, and H.J. Laanbroek. FEMS Microbiol. Ecol. 1998, 27:339-350.
8. Braker, G., H.L. Ayala-del-Rio, A.H. Devol, A. Fesefeldt, and J.M. Tiedje, Appl. Environ. Microbiol. 2001, 67:1893-1901.
9. Stahl, D.A., Mol. Ecol., 1995, 4:535-542.
10.Rooney-Varga. J.N., R. Devereux, R.S. Evans, and M.E. Hines. Appl. Environ. Microbiol., 1997, 63:3895-3901.
11. Weber, S., S. Stubner, and R. Conrad, Appl. Environ. Microbiol, 2001, 67:13181327.
12.Sahm, K., B.J. MacGregor, B.B. Jorgensen, and D.A. Stahl. Environ. Microbiol, 1999, 1:65-74.
13.Zehr, J.P., M. Mellon, S. Braun, W. Litaker, T. Steppe, and H.W. Paerl, Appl. Environ. Microbiol, 1995, 61:2527-2532.
14.Rotthauwe, J.H., K.P. Witzel, and W. Liesack,, Appl. Environ. Microbiol, 1997, 63:4704-4712.
15.Braker, G., A. Fesefeldt, and K.-P. Witzell, Appl. Environ. Microbiol, 1998, 64:3769-3775.
16.Scala, D.J., and L.J. Kerkhoff. Appl. Environ. Microbiol, 2000, 66:1980-1986.
17.Braker, G., J. Zhou, L. Wu, A.H. Devol, and J,M. Tiedje, Appl. Environ. Microbiol, 2000, 66:2096-2104.
18.Henckel, T., P. Roslev, and R. Conrad, Environ. Microbiol., 2000, 2:666-679.
19.Smith, G.B. and J.M. Tiedje. Appl. Environ. Microbiol, 1992, 58:376-384.
20.Small, J., D.R. Call, F.J. Brockman, T.M. Straub, and D. P. Chandler. Appl. Environ. Microbiol, 2001, 67:4708-4716. 21.Wilson, K.H., W.J. Wilson, J.L. Radosevich, T.Z. De-Santis, V.S. Viswanthan, T.A. Kuczmarski, and G. L. Andersen. Appl. Environ. Microbiol, 2002, 68:25352541.
22.Shalon, D.S., J. Smith, and P.O. Brown, Genome Res., 1996, 6:639-645.
About Author / Additional Info:
A Researcher with Ph.D. in Molecular Biology and Biotechnology